P53-DNA Recognition: Difference between revisions
No edit summary |
No edit summary |
||
Line 59: | Line 59: | ||
==Hoogsteen vs. Watson-Crick Base pair in p53 Binding Sites== | ==Hoogsteen vs. Watson-Crick Base pair in p53 Binding Sites== | ||
The distinct <scene name='Sandbox_Reserved_170/Arg248/2'>shape of the minor groove recognized by Arg248</scene> is due to a transition of the four A/T base pairs of the CATG core elements to a Hoogsteen base pairing geometry. Regions with Hoogsteen base pairs ( | The distinct <scene name='Sandbox_Reserved_170/Arg248/2'>shape of the minor groove recognized by Arg248</scene> is due to a transition of the four A/T base pairs of the CATG core elements to a Hoogsteen base pairing geometry. Regions with Hoogsteen base pairs (green) <scene name='Sandbox_Reserved_170/Hg_helix/2'>decrease the diameter of the double helix</scene> compared to regions with Watson-Crick base pairs (blue). | ||
The reason for this deformation of the double helix is the <scene name='Group:USC-LCHS/3kz8_ba_hoogsteencloseup/2'>base pairing geometry in Hoogsteen base pairs</scene> with the approximately 180 degree rotation of adenine around the glycosidic bond and formation of hydrogen bonds with thymine at a different edge of the adenine compared to <scene name='Group:USC-LCHS/3kmd_wcbp_closeup/1'>standard Watson-Crick geometry bps</scene>. | |||
Revision as of 04:58, 4 July 2012
This Sandbox is Reserved from 22 April 2012, through 31 August 2012 for use in the course "Protein DNA" taught by Remo_Rohs at the La Canada High School, USA. This reservation includes Sandbox Reserved 169 through Sandbox Reserved 170. |
To get started:
More help: Help:Editing |
This is a joint project of La Canada High School and University of Southern California students, mentored by Professor Remo Rohs.
A Base Pairing Variant Enhances p53 Binding to a Response ElementA Base Pairing Variant Enhances p53 Binding to a Response Element
Introduction and Biological Role of the Tumor Suppressor p53Introduction and Biological Role of the Tumor Suppressor p53
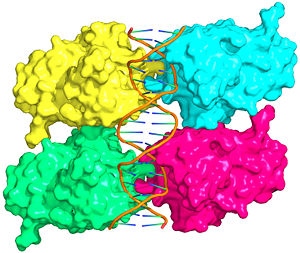
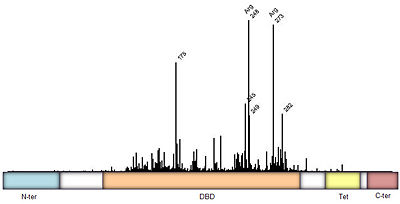
Also known as the Guardian of the Genome, the tumor suppressor p53 is central in the natural defense against human cancer. The protein is activated by stress factors that can compromise the genomic integrity of the cell, and this activation unleashes the function of p53 as transcription factor. It binds as a tetramer (Figure 1) to a large range of DNA response elements. The p53 consensus site (Figure 2) is formed by two decameric half-sites, each containing a core element (red), that are separated by a variable number of base pairs (blue).
Binding of p53 to different response elements leads to distinct biological responses, such as cell-cycle arrest, senescence, or apoptosis. These different pathways correspond, at least in part, to differences in p53-DNA binding affinity and stability, which are determined by specific protein-DNA interactions.
Mutations of p53 residues are associated with 50% of human cancers. Such mutations are predominantly located in the p53-DNA binding domain (DBD) based on an analysis of human tumors (Figure 3). Particularly, arginine residues in the p53-DNA interface were found in tumors with high frequencies.
Structural Description of p53-DNA ComplexStructural Description of p53-DNA Complex
Domain Architecture and TetramerizationDomain Architecture and Tetramerization
|
|
The p53 protein consists of the N-terminal transactivation, the DNA binding or core, the tetramerization, and the C-terminal regulatory domain (Figure 3). This Proteopedia page discusses protein-DNA recognition by p53, thus focuses on the DBD of p53. The only other domain for which structural information is available is the , which forms as a dimer of dimers with one alpha helix and one beta strand contributed by each p53 monomer.
The , which consists of two half sites. These decameric half sites can be separated by a spacer of flexible length but in this case the spacer is of length zero base pairs. The with each dimer binding to one half site of the response element.
The p53 DBD assumes the conformation of an , which binds the response element in the major groove. A functionally important and, thus, stabilizes the fold of the DBD.
Protein-Protein InteractionsProtein-Protein Interactions
The p53 tetramer forms a relatively small and, in comparison, a large . The actual molecular interactions and strength in binding can vary as a function of the sequence and spacer length of the response element.
Major Groove Base ReadoutMajor Groove Base Readout
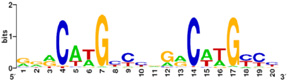
Protein side chains and base pairs form direct contacts in the major groove among which the contributes most to binding specificity. This highly specific readout is due to the . As a result of this base readout the identity of the G/C base pairs in the CWWG core elements is the most conserved position in p53 response elements (Figure 5).
Another important contact is formed with the . Lys120 is biologically very important because acetylation of this residue is known to trigger the apoptotic response.
DNA Backbone ContactDNA Backbone Contact
Another arginine residue, and seems to be important for p53-DNA binding.Moreover, Arg273 is the second most common missense mutation in human cancer (Figure 2).
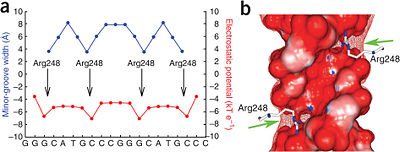
Minor Groove Shape ReadoutMinor Groove Shape Readout
Most commonly, however, the residue Arg248 is found mutated in human tumors. although it does not usually form hydrogen bonds with the bases. Arg248 was shown to recognize regions of narrow minor groove associated with enhanced negative electrostatic potential[1]. This observation provides a novel molecular explanation of the importance Arg248, the most frequently mutated residue in cancer, for p53-DNA binding. This mechanism known as shape readout was found to be broadly employed by arginine residues[3].
Hoogsteen vs. Watson-Crick Base pair in p53 Binding SitesHoogsteen vs. Watson-Crick Base pair in p53 Binding Sites
The distinct is due to a transition of the four A/T base pairs of the CATG core elements to a Hoogsteen base pairing geometry. Regions with Hoogsteen base pairs (green) compared to regions with Watson-Crick base pairs (blue).
The reason for this deformation of the double helix is the with the approximately 180 degree rotation of adenine around the glycosidic bond and formation of hydrogen bonds with thymine at a different edge of the adenine compared to .
Further ReadingFurther Reading
A more general discussion of structural origins of binding specificity in protein-DNA recognition has been published along with a suggestion for a new classification of protein-DNA readout modes that goes beyond the historical description of direct and indirect readout[4].
AcknowledgementsAcknowledgements
This Proteopedia page originates from the partnership of the Rohs Laboratory at the University of Southern California with La Canada High School. This partnership was initiated by Remo Rohs and Patty Compeau in September 2011 as Bioinformatics Institute, which is part of the Institutes of the 21st Century. Contributors to this page are USC graduate students Ana Carolina Dantas Machado, Proteopedia editor Eran Hodis, and La Canada High School students (xxx). Research presented in this article has been conducted in the Shakked Lab at the Weizmann Institute of Science, the Rohs and L. Chen Labs at USC, and the Honig Lab at Columbia University. Furthermore, technical help by Proteopedia editors Eran Hodis, Eric Martz, Jaime Prilusky, and Joel Sussman is acknowledged.
ReferencesReferences
- ↑ 1.0 1.1 1.2 1.3 Kitayner M, Rozenberg H, Rohs R, Suad O, Rabinovich D, Honig B, Shakked Z. Diversity in DNA recognition by p53 revealed by crystal structures with Hoogsteen base pairs. Nat Struct Mol Biol. 2010;17(4):423-9.
- ↑ Jeffrey PD, Gorina S, Pavletich NP. Crystal structure of the p53 tetramerization domain. Science 1995;267:1498-502.
- ↑ Rohs R, West SM, Sosinsky A, Liu P, Mann RS, Honig B. The role of DNA shape in protein-DNA recognition. Nature. 2009;461(7268):1248-53.
- ↑ Rohs R, Jin X, West SM, Joshi R, Honig B, Mann RS. Origins of specificity in protein-DNA recognition. Annu Rev Biochem. 2010;79:233-69.