User:R. Jeremy Johnson/RNaseA: Difference between revisions
No edit summary |
No edit summary |
||
Line 13: | Line 13: | ||
The amino acid sequence was discovered to determine the three-dimensional structure of RNase A by Christian Anfinsen in the 1950s. Urea was used to denature RNase A, and mercaptoethanol was used to reduce and cleave the four disulfide bonds in RNase A to yield eight Cys residues. Catalytic activity was lost due to denaturation. When the urea and mercaptoethanol were removed, the denatured ribonuclease refolded spontaneously into its correct tertiary structure with restoration of its catalytic activity. Disulfide bonds were also reformed in the same position. The Anfinsen experiment provided evidence that the amino acid sequence contained all the information required for the protein to fold into its native three-dimensional structure. Anfinsen received the 1972 Nobel Prize in Chemistry for his work with RNase A. Nevertheless, ensuing work showed some proteins require further assistance, such as molecular chaperones, to fold into their native structure. | The amino acid sequence was discovered to determine the three-dimensional structure of RNase A by Christian Anfinsen in the 1950s. Urea was used to denature RNase A, and mercaptoethanol was used to reduce and cleave the four disulfide bonds in RNase A to yield eight Cys residues. Catalytic activity was lost due to denaturation. When the urea and mercaptoethanol were removed, the denatured ribonuclease refolded spontaneously into its correct tertiary structure with restoration of its catalytic activity. Disulfide bonds were also reformed in the same position. The Anfinsen experiment provided evidence that the amino acid sequence contained all the information required for the protein to fold into its native three-dimensional structure. Anfinsen received the 1972 Nobel Prize in Chemistry for his work with RNase A. Nevertheless, ensuing work showed some proteins require further assistance, such as molecular chaperones, to fold into their native structure. | ||
=Ribonuclease A Catalysis= | ==Ribonuclease A Catalysis== | ||
=Introduction= | |||
[[Image:RNaseAIII.png|300px|left|thumb|Figure I: Bovine Ribonuclease A. Colored residues are representative of amino acids important to both the acid base catalysis (Red: His12 and 119) and stabilization of the transition state (Blue: Lys41). Figure generated via ''Pymol'' ]] | [[Image:RNaseAIII.png|300px|left|thumb|Figure I: Bovine Ribonuclease A. Colored residues are representative of amino acids important to both the acid base catalysis (Red: His12 and 119) and stabilization of the transition state (Blue: Lys41). Figure generated via ''Pymol'' ]] | ||
Revision as of 21:58, 18 May 2011
|
IntroductionIntroduction
|
Ribonucleases [1] or RNA depolymerases are enzymes that catalyze RNA degradation. Ribonucleases are highly active in ruminants [2], such as cows, to digest large amounts of RNA produced by microorganisms in the stomach. Ruminants also have high amounts of ribonucleases to process nutrients from cellulose. One such ribonuclease, bovine ribonuclease A or RNase A, is a model enzyme due to its ease of purification and simple structure.
RNase A has been used as a foundation enzyme for study due to its stability, small size, and because its three-dimensional structure is fully determined by its amino acid sequence, needing no molecular chaperones. The 1972 Nobel Prize in Chemistry was awarded to three researchers for their work with RNase A on the folding of chains in RNase A and the stability of RNase A. The previously mentioned Christian Anfinsen received the 1972 Nobel Prize in Chemistry for his paper "Principles that govern the folding of protein chains." Stanford Moore and William H. Stein received the 1972 Nobel Prize in Chemistry for their paper "The chemical structures of pancreatic ribonuclease and deoxyribonuclease." The 1984 Nobel Prize in Chemistry was awarded to Robert Bruce Merrifield for his paper "Solid-phase synthesis" using RNase A (Raines). RNase A was the first enzyme and third protein for which its amino acid sequence was correctly determined and the third enzyme and fourth protein whose three-dimensional structure was determined by X-ray diffraction analysis [3]. Disulfide bonds in RNase A were determined after developing a method using Fast Atom Bombardment Mass Spectrometry (FABMS) [4]. The methods of NMR spectroscopy [5] and Fourier transform infrared (FTIR) spectroscopy [6] were developed with RNase A in determining protein structure and protein folding pathways. These new methods, developed with RNase A, could be used for further research to determine the protein structure and protein folding pathways of other proteins (Raines).
StructureStructure
RNase A is made up of a single polypeptide chain of 124 residues. Of the 20 natural amino acids, RNase A possesses 19 of them, excluding tryptophan. This single polypeptide chain is cross-linked internally by four , which contribute to the stability of RNase A. Long four-stranded anti-parallel and three short make up the of RNase A (Raines). The structure of RNase A is often described as kidney shaped, with the active-site residues located within the cleft. residues aid in catalysis. stabilizes the negative charge in the transition state, while acts as a base and acts as an acid in catalysis.
The amino acid sequence was discovered to determine the three-dimensional structure of RNase A by Christian Anfinsen in the 1950s. Urea was used to denature RNase A, and mercaptoethanol was used to reduce and cleave the four disulfide bonds in RNase A to yield eight Cys residues. Catalytic activity was lost due to denaturation. When the urea and mercaptoethanol were removed, the denatured ribonuclease refolded spontaneously into its correct tertiary structure with restoration of its catalytic activity. Disulfide bonds were also reformed in the same position. The Anfinsen experiment provided evidence that the amino acid sequence contained all the information required for the protein to fold into its native three-dimensional structure. Anfinsen received the 1972 Nobel Prize in Chemistry for his work with RNase A. Nevertheless, ensuing work showed some proteins require further assistance, such as molecular chaperones, to fold into their native structure.
Ribonuclease A CatalysisRibonuclease A Catalysis
IntroductionIntroduction
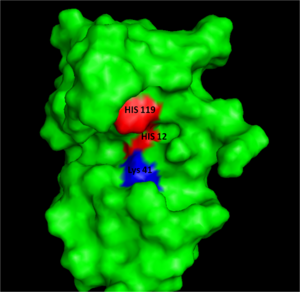
| |||||||||
7rsa, resolution 1.26Å () | |||||||||
---|---|---|---|---|---|---|---|---|---|
Ligands: | , | ||||||||
Activity: | Pancreatic ribonuclease, with EC number 3.1.27.5 | ||||||||
| |||||||||
| |||||||||
| |||||||||
Resources: | FirstGlance, OCA, RCSB, PDBsum | ||||||||
Coordinates: | save as pdb, mmCIF, xml |
Acid Base CatalysisAcid Base Catalysis
In organic chemistry acid/base catalysis is the addition of an acid or base to accelerate a chemical reaction. Ribonuclease A, (RNase A), also uses acid/base catatalysis to chemically change its substrates. Acidic or basic residues of the enzyme transfer protons to or from the reactant in order to stabilize the developing charges in the transition state. The transfer of protons usually creates better leaving groups, making the reaction more energetically favorable. Histidine is a very common amino acid residue involved in cataylsis, as histidine has a pKa value close to neutral, (pKa=6); therefore, histidine can both accept and donate protons at physiological pH.
Acid/base catalysis by an enzyme is dependent on the pH of the environment and the pKa's of their residues. The pKa value will increase for an acidic residue if the environment is hydrophobic or if the adjacent residues are of similar charges. In the same environmental conditions, a basic residue will decrease the pKa. This ability to alter the pKa of certain residues such as histidines, increases the diversity of reactions that an enzyme can perform.
Active Site StructureActive Site Structure
RNase A uses acid/base catlysis to speed up RNA hydrolysis. This occurs in the which is found in the cleft of RNase A and is the location of the chemical change in bound substrates. Subsites lining the active site cleft are important to the binding of single stranded RNA. Large quantities of positively charged residues, such as and and , recognize the negative charge on the phosphate back bone of the RNA strand [1].
The active site for RNase A, although fairly nonspecific, has some specificity for sites RNA hydrolysis. , located next to the active site, will hydrogen bond to pyrimidine bases, but sterically hinder the binding of a purine on the 5' strand of OH. Thr45 significantly decreases the rate of hydrolysis of polymeric purine strands, such as poly A, by a thousand fold, as compared to polymeric pyrimidine strands [1].
Early studies on RNase A catalysis showed that alkylation of His12 and His119 significantly decreased its catalytic activity, prompting the hypothesis that these two histidines were the acid/base catalyst. Confirmation of this hypothesis came when these histidines were replaced with alanine and the reaction rates of either mutation dropped by ten-thousand fold [1].
Acid Base Catalysis by RNase AAcid Base Catalysis by RNase A
MechanismMechanism
RNase A catalyzes the cleavage of the Phosphodiester bonds in two steps: the formation of the pentavalent phosphate transition state and subsequent degradation of the 2’3’ cyclic phosphate intermediate. An important part of the reaction is the ability of histidine (His 12 and His119) to both accept and donate electrons, allowing these histidine to be an acid or a base, making the reaction pH dependent [2].

RNA hydrolysis begins when abstracts a proton from the 2’ OH group on RNA; thus, assisting in the nucleophilic attack of the 2’ oxygen on the electrophilic phosphorus atom. A transition state is then formed, having a pentavalent phosphate, which is stabilized by the positively charged amino group of and the main chain amide nitrogen of Phe120. then protonates the 5' oxygen on the ribose ring and the transition state falls to form a 2’3’cyclic phosphate intermediate [2].
In a secondary and separate reaction, the 2’,3’ cyclic phosphate is hydrolyzed to a mixture of 2'phosphate and 3' hydroxyl. His12 donates a proton to the leaving group of this reaction, the 3’ oxygen of the cyclic intermediate. Simultaneously, His-119 abstracts the proton from a water molecule, activating it for nucleophilic attack. The activated water molecule attacks the cyclic phosphate causing the cleavage of the 2'3’ cyclic phosphate intermediate. The truncated nucleotide is then released with a 3’ phosphate group [2].
InhibitorsInhibitors
Due to the highly catalytic nature of RNase A for RNA strands, mammalian cells have developed a protective inhibitor to prevent pancreatic ribonucleases from degrading cystolic RNA. Ribonuclease Inhibitor (RI) tightly associates to the active site due to its non-globular nature. RI is a large 50 kD protein that is composed of 16 repeating alpha and beta chains, giving it a noticable horseshoe like appearance. It has been suggested that RI has the highest protein-protein interactions with an approximate dissociation constant (Kd) of 5.8 X 10-14 for almost all types of RNases [3].
The ability to be selective for almost all types of RNases, and yet retain such a high Kd is product of its mechanism. The interior residues of the horseshoe shaped RI are able to bind to the charged residues of the active site cleft of RNase A, such as Lys7, Lys9, Lys 41 and Gln11. By studying the amphibian RNase, Onconase, the residues Lys7 and Gln11 of RNase A were shown to be the most important in this interaction. In onconase, these residues are replaced with non-charged amino acids, which help prevent the binding of RI to the protein [4].
Evolutionary SignificanceEvolutionary Significance
RNase variants have undergone duplication six times since amphibians and mammals diverged, giving rise to RNase A and other homologues. RNase A was believed to have become more specified within bovids[7] 35 million years ago (Opitz et al. 1997). RNase A homologues have been found in frogs and humans by comparing the amino acid sequences of these particular enzymes with RNase A and seeing what residues were conserved. , shown here for the homologues of RNase A, can either support or refute theories of protein structure and function. There have been over 40 different RNase homologues that have been sequenced. Conservation of amino acids Lys41 and His12 and His119 maintain the catalytic function within RNase A homologues. However, these RNase A homologues differ in cytotoxicity and also have slight differences in sequences which may lead to different functions. One homologue, angiogenin, promotes neovascularization [8]. Unusual homologues include other RNase homologues in the human body such as in urine and red blood cells. (Raines)
Ribonuclease A Substrate BindingRibonuclease A Substrate Binding
IntroductionIntroduction
|
RNase A is an endonuclease that cleaves and breaks down RNA using acid base catalysis. RNase A has been a model protein for studies on the stability, folding and chemistry of proteins. ‘[5]’ It is also essential in protein regulation within the body due to its function of RNA degradation.
Substrate BindingSubstrate Binding

To determine the structural characteristics of RNA substrate binding to RNase A, X-ray crystallography was used to image inhibitory DNA tetramers bound to RNase A. DNA lacks the 2’OH essential to RNA cleavage, making the complex more conducive to crystallography. between RNase A and (1rta) provides information about specificity of the binding pocket subunits, B0, B1, B2 and B3. Many interactions observed in this complex occur between amino acid residues and the nucleic acid backbone. Examples of these interactions include hydrogen bonding between as well as hydrogen bonding between the O5’ oxygen of the ribose of . ‘[6]’

Further binding pocket characterization was performed using (1rcn). This was important in determining the specificity of the binding sites of RNase A. In this complex, the B1 site is thought to exclusively bind to pyrimidine bases due to steric interactions and . When Thr45 was mutated to glycine, purines readily bound to the B1 site. ‘[7]’ This interaction appears to be the driving force behind its inability to bind purines. While binding of other nucleobases to the B2 and B3 sites is possible, the imaging of this complex elucidated the preferences for adenosine bases at these two positions. In addition to its catalytic activity His119 has also been shown to be important in substrate specificity. This is due to the . When this site was mutated, the affinity for a poly(A) substrate was decreased by 104-fold. ‘[8]It also establishes hydrogen bonding between . ‘[9]’
ConclusionConclusion
From the studies on RNase A complexes with deoxy nucleic acid tetramers, it has been established that this enzyme recognizes the substrate on both its phosphate backbone and on individual nucleobases. RNase A has a nonspecific B0 site, a B1 site specific to pyrimidines and a B3 and B4 site with a preference for adenosine bases. Similar to other enzymes, RNase A uses the hydrogen bonding distance between amino acids and the substrate to bind specifically to certain nucleobases. Studying the substrate recognition and specificity of enzymes such as RNase A is an important step in understanding the regulation of RNA within biological systems.
Medical ImplicationsMedical Implications
A recent study by Patutina et al. (2011) revealed that tumor propagation is associated with an imbalance in nucleic acid metabolism. In the blood plasma of patients, there were increased levels of circulating nucleic acids and decreased nuclease activity. The abnormally high levels of circulating nucleic acids were associated with the increased expression and secretion of tumor-derived miRNA and DNA. With increased expression, the tumor progresses and the patient has a bad prognosis.
RNase A and DNase I inhibit metastasis [9] by catalyzing metastasis pathomorphosis which is apoptosis, necrosis [10] and destruction of oncocytes [11]. This capability retards the primary tumor growth by 30-40%. The tumor bearing mice received doses of RNase A, DNase I or a mixture of the two and the most significant effect observed was in the mice treated with both enzymes simultaneously. Thus the simultaneous administration of RNase A and DNase I led to an anti-metastatic effect and resulted in an almost complete absence in the metastasis of the tumor. Further observations suggest that RNase A and DNase I are toxic at high levels. So for effective treatment, ultra low doses are required to stay below the level of toxicity.
Another member in the ribonuclease family and structural homologue to bovine RNase A is frog onconase [12] or ONC. ONC is found in oocytes [13] and early embryos of northern leopard frogs. The frog ribonuclease variant shows both cytostatic (cell growth suppression) and cytotoxic (prevents cell divisions) characteristics when it interacts with tumor cells. According to Gahl et al. (2008), no side effects have been determined for ONC. Leland et al. (2001) looked to determine the interactions that control the folding of ONC in order to develop effective mimics of ONC. In order to determine the interactions that controlled folding, the regeneration of RNase A was studied. Although RNase A and ONC were structurally very similar, there were significant differences in their folding pathways. While ONC forms a stable disulfide intermediate, RNase A does not. ONC was also found to be missing a disulfide bond that RNase A possesses. In the case of both enzymes, entropy is lost in the formation of the disulfide bonds, but folding may be driven by enthalpically favorable interactions of the side chains. Further experiments are being done to identify intramolecular interactions that account for the increased rate and formation of the structured intermediate in ONC (Gahl).
ReferencesReferences
- ↑ 1.0 1.1 1.2 Cite error: Invalid
<ref>
tag; no text was provided for refs namedWlodrawer1988
- ↑ 2.0 2.1 2.2 Cite error: Invalid
<ref>
tag; no text was provided for refs namedRaines1998
- ↑ Cite error: Invalid
<ref>
tag; no text was provided for refs namedVicentini1996
- ↑ Cite error: Invalid
<ref>
tag; no text was provided for refs namedTurcotte2008
- ↑ Raines RT. Ribonuclease A. Chem Rev. 1998 May 7;98(3):1045-1066. PMID:11848924
- ↑ Birdsall DL, McPherson A. Crystal structure disposition of thymidylic acid tetramer in complex with ribonuclease A. J Biol Chem. 1992 Nov 5;267(31):22230-6. PMID:1429575
- ↑ delCardayre SB, Raines RT. Structural determinants of enzymatic processivity. Biochemistry. 1994 May 24;33(20):6031-7. PMID:8193116
- ↑ Thompson JE, Raines RT. Value of general Acid-base catalysis to ribonuclease a. J Am Chem Soc. 1994 Jun;116(12):5467-8. PMID:21391696 doi:10.1021/ja00091a060
- ↑ Fontecilla-Camps JC, de Llorens R, le Du MH, Cuchillo CM. Crystal structure of ribonuclease A.d(ApTpApApG) complex. Direct evidence for extended substrate recognition. J Biol Chem. 1994 Aug 26;269(34):21526-31. PMID:8063789
External ResourcesExternal Resources
- Full crystal structure of 1RTA
- Full crystal structure of 1RCN
- Further information on 1RTA
- RNase A- Molecule of the Month
http://en.wikipedia.org/wiki/RNase_A
http://en.wikipedia.org/wiki/Ribonuclease_inhibitor
Literary CitationsLiterary Citations
Ferreri, Carla; Chryssostomos Chatgillaloglu, Armida Torreggiani, Anna Marla Salzano, Giovanni Rensone, and Andrea Scaloni. “The Reductive Desulfurization of Met and Cys Residues in Bovine RNase A Is Assoicated with trans Lipid Formation in a Mimetic Model of Biological Membranes.” Journal of Proteom. 7 (2008): 2007-2015[14]
Gahl, R. F. et al. “Dissimilarity in the oxidative folding of onconase and ribonuclease A, two structural homologues.”Proetin Engineering, Design & Selection. 21 (2008) 223-231[15]
H. P. Avey; M. O. Boles; C. H. Carlisle; S. A. Evans; S. J. Morris; R. A. .Palmer; B. A. Woolhouse.”Structure of Ribonuclease.” Nature. 213 (1967) 557-562[16]
H. W. Wyckoff, Karl D. Hardman; N. M. Allewell; Tadash Inagam; L. N. Johnson. “The Structure of Ribonuclease-S at 3.5 A Resolution.” Department of Molecular Biophysics, Yale University. 242 (1967): 3984-3988 [17]
Kadonosono, Tetsuya; Eri Chatani, Rikimaru Hayashi, Hideaki Moriyama, and Tatzuo Ueki. “Minimization of Cavity Size Ensures Protein Stability and Folding: Structures of Phe-46-Replaced Bovine Pancreatic RNase A.” Biochemistry. 42 (2003): 10651-10658 [18]
Nelson, L. D., M. Cox. "Lehninger Principles of Biochemistry" New York, NY. 2008 (Fifth Edition)
Opitz, J. G. et al. “Origin of the catalytic activity of bovine seminal ribonuclease against double-stranded RNA.” Biochemistry 1998. 37 (4023-4033)
Patutina, Olga; Nadezda Mironova, Elena Ryabchikova, Nelly Popova, Valery Nikolin, Vasily Kaledin, Valentin Valssov, Marina Zenkova. “ Inhibition of Metastasis Development by Daily Administration of Ultralow Doses of RNase A and DNase I” Biochimie. 93 (2011) 689-696 [19]
Raines, Ronald T. “Ribonuclease A.” Chemistry Review. Madison Wisconsin. 98 (1998): 1045-1065 [20]
Wlodawer, Alexander; L. Anders Svensson, Lennart Sjolin, Gary L. Gilliland. “Structure of Phosphate-Free Ribonuclease A Refined at 1.26 A.” American Chemical Society. 27 (1988) 2705-2717 [21]